redox processes Notes
Oxidation and reduction
Introduction to oxidation and reduction
- The term oxidation has been used to describe reactions where oxygen is involved in chemical change.
- Oxidation, though, is only half of the story, as it is always accompanied by the opposite process reduction, which was originally thought of in terms of loss of oxygen.
Oxidation | Reduction |
gain of oxygen | loss of oxygen |
loss of hydrogen | gain of hydrogen |
Table 1: Introduction to oxidation and reduction
- So, oxidation and reduction will always occur together and reactions of this type are known as redox reactions.
Oxidation: Combining with oxygen
- At the simplest level oxidation can be considered as a reaction in which a substance combines with oxygen. Examples include:
2Mg(s) + O2(g) → 2MgO(s)
2CH3OH(l) + 3O2(g) → 2CO2(g) + 4H2O(l)
4Fe(s) + 3O2(g) → 2Fe2O3(s)
- Fe2O3(s), iron(III) oxide, is rust. Rusting is an example of the process of corrosion.
Reduction: Removal of oxygen or addition of hydrogen
- Reduction may be considered as the removal of oxygen, for example:
NiO(s) + C(s) → Ni(s) + CO(g)
- In this reaction nickel(II) oxide is reduced by carbon to give metallic nickel.
- Reduction may also be considered as the addition of hydrogen. An example of such a reaction is:
WO3(s) + 3H2(g) → W(s) + 3H2O(g)
- This mirrors the previous interpretation of reduction, as oxygen is removed from tungsten(VI) oxide in the process.
Oxidation and reduction in terms of electron transfer
- In terms of electron transfer, oxidation and reduction can be defined as follows:
- Oxidation involves the loss of electrons and reduction involves the gain of electrons.
- A useful mnemonic for remembering this is OILRIG:
- Oxidation Is Loss of electrons.
- Reduction Is Gain of electrons.
Oxidation and reduction in terms of oxidation states
- The oxidation state is the apparent charge of an atom in a free element, a molecule, or an ion.
- In terms of oxidation state:
- Oxidation describes a process in which the oxidation state increases and reduction describes a process in which the oxidation state decreases.
Variable oxidation states
- Many elements have fixed oxidation states, in their ions and compounds, such as the group 1 alkali metals (eg +1 for Na) and the group 2 alkaline earth metals (eg +2 for Ca), variable oxidation states exist for many main-group non-metals and in particular for most of the transition elements (also called the transition metals).
- Indeed variable oxidation states are a characteristic property of the transition metals.
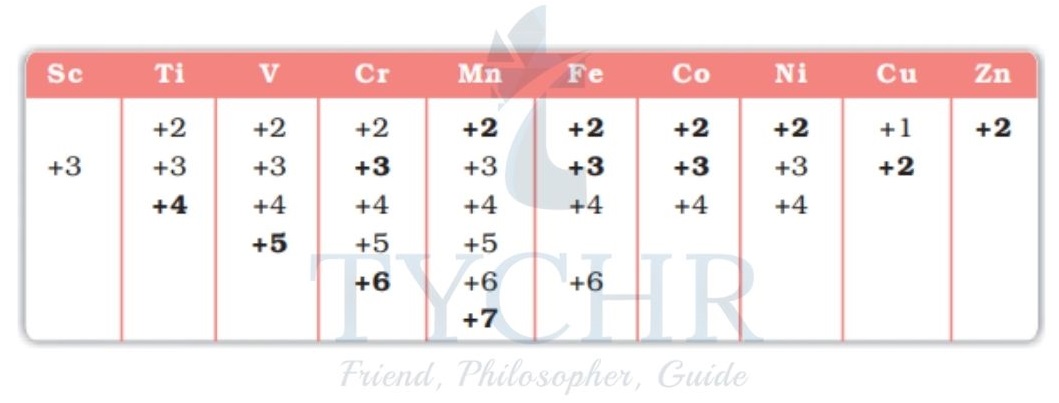
Oxidation states and the nomenclature of transition metal compounds
- The characteristics of transition elements is that they can have variable oxidation states in their compounds.
- Traditionally, the Roman numeral system of nomenclature has been used to name such compounds and this system is based on oxidation numbers.
- The system is called the Stock nomenclature system. In the Stock system, Roman numerals (I, II, III etc.) are used to indicate the oxidation number.
Expressing redox reactions using half-equations in acidic or neutral solutions
- Half-equations can be very useful in balancing complex redox reactions. Each half-equation represents the separate oxidation and reduction processes. The following general working method can be used to balance a redox reaction involving oxidation states.
Working method
- Step 1: Assign oxidation states for each atom in the reactant and product species.
- Step 2: Deduce which species is oxidized and which species is reduced
- Step 3: State the half-equation for the oxidation process and the corresponding half-equation for the reduction process.
- Step 4: Balance these half-equations so that the number of electrons lost equals the number of electrons gained.
- Step 5: Add the two half-equations together to write the overall redox reaction.
- Step 6: Check the total charge on the reactant and product sides.
- Step 7: Balance the charge by adding H+ and H2O to the appropriate sides.
Oxidizing and reducing agents
- Redox reactions always involve the simultaneous oxidation of one reactant with the reduction of another as electrons are transferred between them.
- The reactant that accepts electrons is called the oxidizing agent as it brings about oxidation of the other reactant. In the process it becomes reduced.
- Likewise the reactant that supplies the electrons is known as the reducing agent, because it brings about reduction and itself becomes oxidized.
- Sometimes the terms reductant and oxidant are used in place of reducing agent and oxidizing agent respectively.
- For example, in the reaction where iron (Fe) is extracted from its ore (Fe2O3):
More reactive metals are stronger reducing agents
- Of course not all oxidizing and reducing agents are of equal strength. Some will be stronger than others, depending on their relative tendencies to lose or gain electrons.
- If we immerse zinc in a solution of copper sulfate, a reaction occurs.
- The blue colour of the solution fades, the pinkish-brown colour of copper metal appears and there is a rise in temperature.
- What is happening is that the Cu2+ ions are being displaced from solution as they are reduced by Zn. At the same time Zn dissolves as it is oxidized to Zn2+.
Zn(s) + CuSO4(aq) → ZnSO4(aq) + Cu(s)
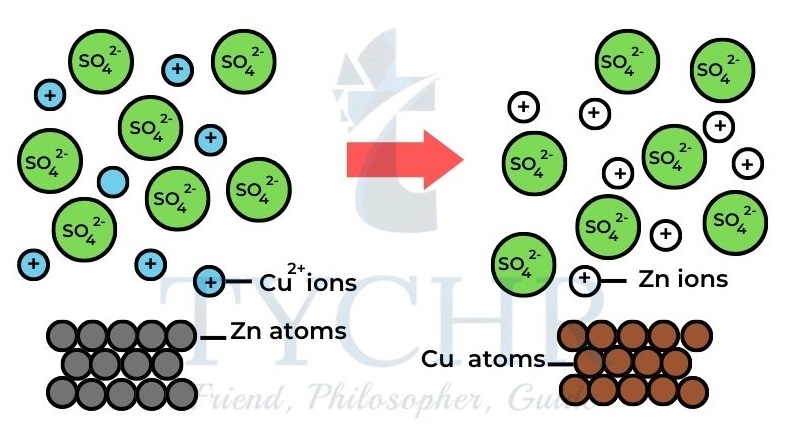
- The activity series ranks metals according to the ease with which they undergo oxidation.
- Metals higher up in the activity series can displace those lower down from solutions of their respective salts.
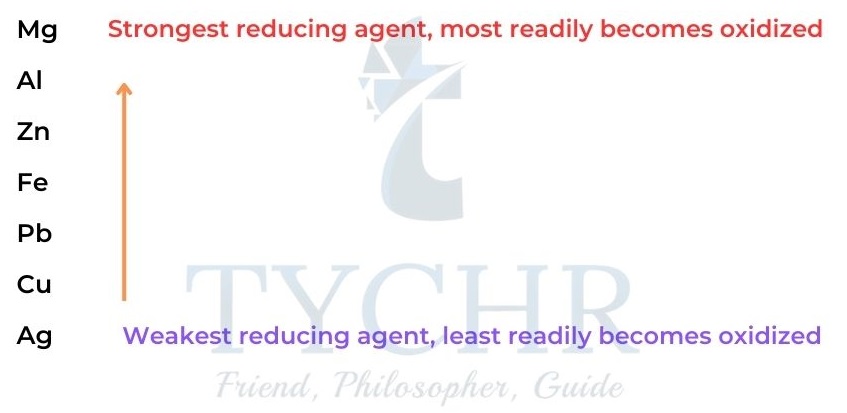
More reactive non-metals are stronger oxidizing agents
- In a similar way, the different strengths of non-metals as oxidizing agents can be compared.
- For example, the halogens (Group 17 elements) react by gaining electrons and forming negative ions, and so act as oxidizing agents by removing electrons from other substances.
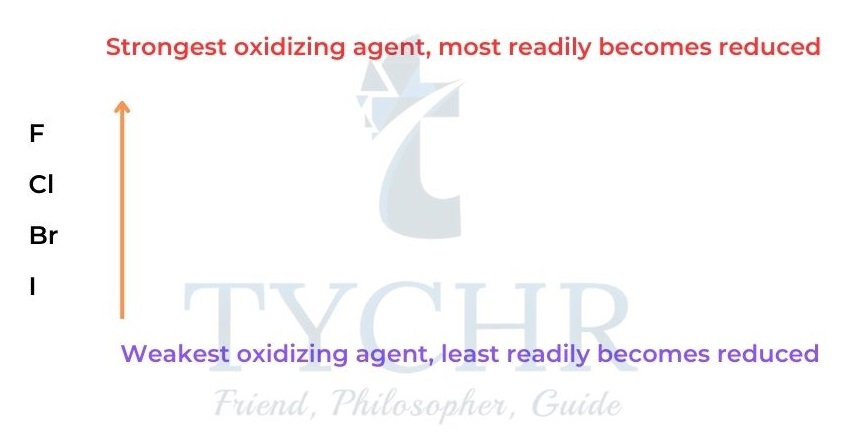
Use of chlorine and ozone as disinfectants in drinking water
- Water supplies are disinfected using strong oxidizing agents such as chlorine, Cl2 or ozone, O3 to kill microbial pathogens.
- Chlorine can be added in three forms: chlorine gas, Cl2; sodium hypochlorite, NaOCl; and calcium hypochlorite, Ca(OCl)2. All three of these solutions yield hypochlorous acid, HOCl, which is the antibacterial agent.
Ozone | Chlorine |
can be used to treat viruses | cannot be used to treat viruses |
leaves no unpleasant residual taste or odour | leaves a residual taste and unpleasant odour |
fewer toxic by-products | can form toxic by-products, often carcinogenic |
more expensive | cheaper |
Table 2: Advantages and disadvantages of using ozone and chlorine in the treatment of water supplies
Redox titrations
Acid–base titration | Redox titration |
neutralization reaction between acid and base | redox reaction between oxidizing agent and reducing agent |
protons are transferred from acid to base | electrons are transferred from reducing agent to oxidizing agent |
Table 3: Comparison of Acid-base titration and Redox titration.
Working method
- Step 1: Deduce the balanced redox equation, using oxidation states.
- Step 2: From the information given, state which three pieces of data are given from VA, cA, VB, and cB and identify the fourth variable that needs to be determined. Identify the stoichiometry coefficients νA and νB from the balanced equation.
- Step 3: Set up the following expression and fill in the known data:
1 /νA (VA × cA) = 1/νB (VB × cB)
- Step 4: Solve for the unknown variable (VA, cA, VB, or cB).
- Step 5: Answer any riders to the question (such as expressing a concentration in particular units).
Electrochemical cells
Energy
- Energy is the capacity to do work. The SI unit of energy is the joule (J).
- The law of conservation of energy states that energy cannot be created or destroyed but is converted from one form to another.
Electrochemical cells
- In an electrochemical cell chemical energy–electrical energy conversions take place, which can go in either direction. There are two main types of electrochemical cell:
- 1) Voltaic (or galvanic) cells – these convert chemical energy to electrical energy. Voltaic cells convert energy from spontaneous, exothermic chemical processes to electrical energy.
- 2) Electrolytic cells – these convert electrical energy to chemical energy, bringing about a non-spontaneous process.
Figure 5: Voltaic cells convert energy from spontaneous exothermic chemical processes to electrical energy. Figure 6: Electrolytic cells convert electrical energy to chemical energy, bringing about a nonspontaneous process.
Electrode
- Electrons are carriers of electric charge in metals. An electrode is a conductor of electricity used to make contact with a non-metallic part of a circuit, such as the solution in a cell (the electrolyte).
- An electrochemical cell contains two electrodes, the anode and the cathode.
- In both voltaic and electrolytic cells:
- oxidation always takes place at the anode
- reduction always takes place at the cathode.
- The polarity of the electrodes differs in the different types of cell.
- In a voltaic cell:
- the cathode is the positive electrode
- the anode is the negative electrode.
- In an electrolytic cell:
- the cathode is the negative electrode
- the anode is the positive electrode.
Voltaic cells
Half-cells generate electrode potentials
- There are many types of half-cell but probably the simplest is made by putting a strip of metal into a solution of its ions.
- In the zinc half-cell, zinc atoms will form ions by releasing electrons that will make the surface of the metal negatively charged with respect to the solution.
- There will therefore be a charge separation, known as an electrode potential, between the metal and its ions in solution.
Figure 8: The zinc half-cell develops a negative potential with respect to the copper half-cell. - In general the more reactive a metal, the more negative its electrode potential in its half-cell.
Two connected half-cells make a voltaic cell
- If we now connect these two half-cells by an external wire, electrons will have a tendency to flow spontaneously from the zinc half-cell to the copper half-cell because of their different electrode potentials.
- The half-cells connected in this way are often called electrodes, and their name gives us information about the type of reaction that occurs there.
- The electrode where oxidation occurs is called the anode, in this case it is the zinc electrode and it has a negative charge: Zn(s) → Zn2+(aq) + 2e–
- The electrode where reduction occurs is called the cathode, in this case it is the copper electrode and it has a positive charge: Cu2+(aq) + 2e– → Cu(s)
- A potential difference will, however, only be generated between the electrodes when the circuit is complete.
- Oxidation always occurs at the anode; reduction always occurs at the cathode. In the voltaic cell, the anode has a negative charge and the cathode has a positive charge.
- The voltaic cell therefore must have the following connections between the half-cells.
- An external electronic circuit, connected to the metal electrode in each half-cell. A voltmeter can also be attached to this external circuit to record the voltage generated. Electrons will fl ow from the anode to the cathode through the wire.
- A salt bridge completes the circuit. The salt bridge is a glass tube or strip of absorptive paper that contains an aqueous solution of ions. Movement of these ions neutralizes any build-up of charge and maintains the potential difference. Anions move in the salt bridge from the cathode to the anode, which opposes the flow of electrons in the external circuit. Cations move in the salt bridge from the anode to the cathode.
- The solution chosen is often aqueous NaNO3 or KNO3 as these ions will not interfere with the reactions at the electrodes. Without a salt bridge, no voltage is generated.
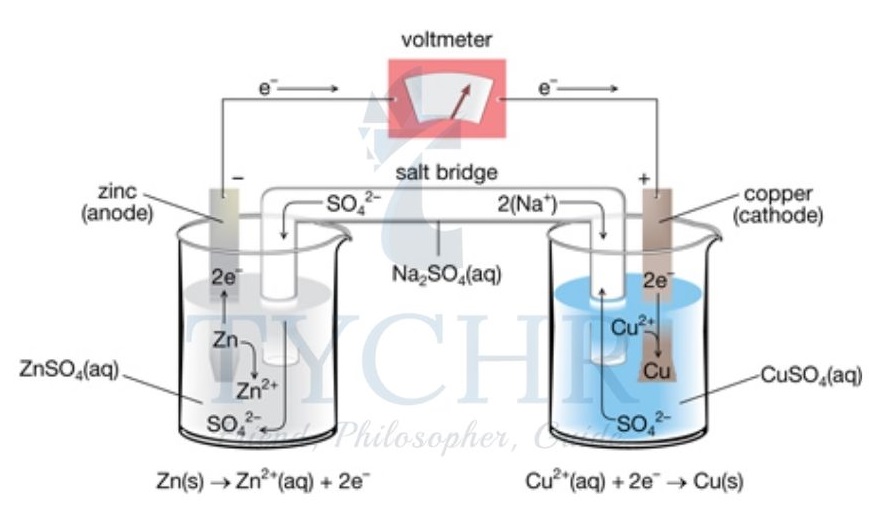
- A voltaic cell converts the energy released from a spontaneous, exothermic reaction into electrical energy.
Different half-cells make voltaic cells with different voltages
- Any two half-cells can be connected together similarly to make a voltaic cell. For any such cell the direction of electron flow and the voltage generated will be determined by the difference in reducing strength of the two metals.
- In most cases this can be judged by the relative position of the metals in the reactivity series. For example if we changed the copper half-cell in the example above to a silver half-cell, a larger voltage would be produced because the difference in electrode potentials of zinc and silver is greater than between that of zinc and copper. Electrons would flow from zinc (anode) to silver (cathode).
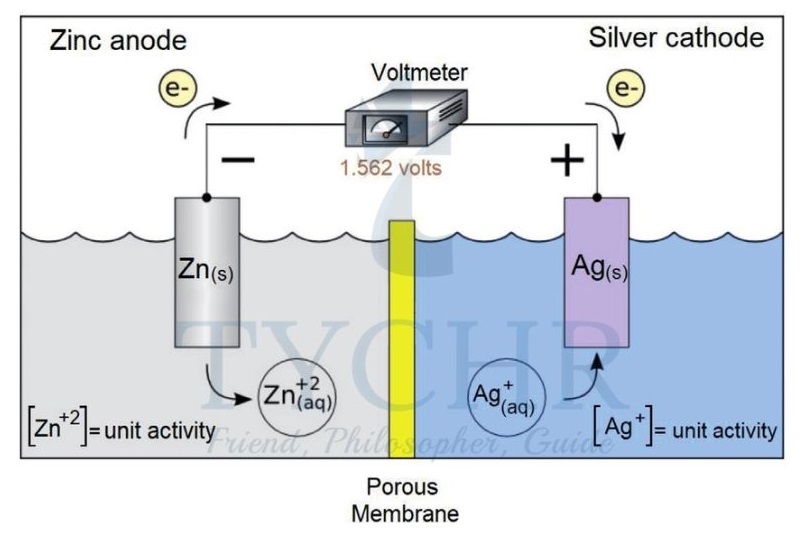
- Electrons always flow in the external circuit from anode to cathode.
- We can now summarize the parts of a voltaic cell and the direction of movement of electrons and ions:
- electrons flow from anode to cathode through the external circuit;
- anions migrate from cathode to anode through the salt bridge;
- cations migrate from anode to cathode through the salt bridge.
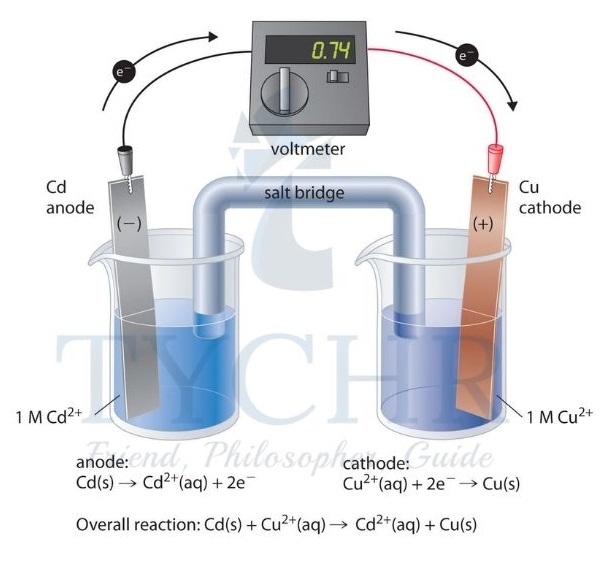
Measuring standard electrode potentials
- As electrode potentials depend on the concentrations of ions, gas pressures, purity of substance, and temperature, these must all be controlled in order to make valid comparisons between different half-cells. So standard conditions are used in these measurements, defined as follows:
- all solutions must have a concentration of 1.0 mol dm–3;
- all gases must be at a pressure of 100 kPa;
- all substances used must be pure;
- temperature is 298 K;
- if the half-cell does not include a solid metal, platinum is used as the electrode.
- Half-cells under these conditions are known as standard half-cells.
- When the standard hydrogen electrode is connected to another standard half-cell by an external circuit with a high-resistance voltmeter and a salt bridge, the EMF generated is known as the standard electrode potential of that half-cell. It is given the symbol E*.
- The positive value for E* indicates that this half-cell has a greater tendency to be reduced than H+. So electrons tend to flow from the hydrogen half-cell, which is therefore oxidized, to the copper half-cell, which is reduced.
Calculating the cell potential, E*cell
- The half-cell with the higher E * value will be reduced, and the half-cell with the lower E * value will be oxidized. As the cell potential is the difference in the tendencies of these two half-cells to be reduced, we can calculate it by substituting the appropriate values into the following expression:
E *cell = E *half-cell where reduction occurs – E *half-cell where oxidation occurs
Determining spontaneity of a reaction
- As E* values can be used to predict the redox change that will occur among a mixture of reactants, it follows we can use them to determine whether a particular reaction will occur spontaneously.
- This is based on the fact that a voltaic cell will always run in the direction that gives a positive value for the E* cell.
- If E* cell is positive, the reaction is spontaneous as written.
- If E* cell is negative, the reaction is non-spontaneous, and the reverse reaction is spontaneous.
Electrode potential and free energy change (Ecell and ∆G)
- We have now come across two quantitative measures of the spontaneity of a reaction, E*cell and the free energy, ∆G*.
- These two values are directly related through the equation:
∆G* = – nFE*
- where n = number of moles of electrons transferred in the reaction;
- F = the charge carried by 1 mole of electrons, known as the Faraday constant (it has a value of approximately 96 500 C mol–1).
- The units of the terms in this equation confirm the relationship:
∆G*(J) = –n (mol) F (C mol–1) E* (V) as J = C × V
- The negative sign in the equation indicates that E and ∆G have opposite signs:
- when E*cell is positive, ∆G* is negative ⇒ reaction is spontaneous;
- when E*cell is negative, ∆G* is positive ⇒ reaction is non-spontaneous;
- when E*cell is 0, ∆G* is 0 ⇒ reaction is at equilibrium.
Electrolytic cells
An external source of electricity drives non-spontaneous redox reactions
- An electrolytic cell does the reverse: it uses an external source of electrical energy to bring about a redox reaction that would otherwise be nonspontaneous.
- The reactant in the process of electrolysis is present in the
- This is a liquid, usually a molten ionic compound or a solution of an ionic compound. As the electric current passes through the electrolyte, redox reactions occur at the electrodes, removing the charges on the ions and forming products that are electrically neutral.
- The ions are therefore said to be discharged during this process.
- The components of an electrolytic cell are:
- The source of electric power is a battery or a DC power source.
- This is shown in diagrams as |I where the longer line represents the positive terminal and the shorter line the negative terminal.
- The electrodes are immersed in the electrolyte and connected to the power supply. They must not touch each other! Electrodes are made from a conducting substance – generally a metal or graphite. They are described as inert when they do not take part in the redox reactions.
- Electric wires connect the electrodes to the power supply. The power source pushes electrons towards the negative electrode where they enter the electrolyte.
- This is the cathode. Electrons are released at the positive terminal, the anode, and returned to the source.
- The current is passed through the electrolyte, not by electrons but by the ions as they are mobile and migrate to the electrodes.
- The chemical reactions occurring at each electrode remove the ions from the solution and so enable the process to continue.
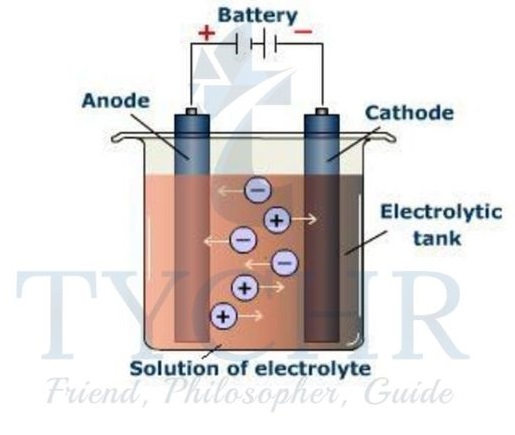
Redox reactions occur at the electrodes
- The ions in the electrolyte migrate to the electrodes by attraction of opposite charges. So positive ions (cations) are attracted to the negative electrode, the cathode, while negative ions (anions) are attracted to the positive electrode, the anode.
- At the electrodes, redox reactions occur, oxidation at the anode and reduction at the cathode, which result in the ions being discharged.
- In aqueous solutions, water can be oxidized to oxygen at the anode and reduced to hydrogen at the cathode.
- At the negative electrode (cathode): M+ + e– → M; cations gain electrons so are reduced.
- At the positive electrode (anode): A– → A + e–; anions lose electrons so are oxidized.
Determining the products in electrolytic cells
We can apply our knowledge of redox chemistry to electrolytic cells, determining the reactions that occur at the electrodes, and so predicting the products released. The following steps are a useful summary:
- Identify all the ions present in the electrolyte and determine which will migrate to which electrode: anions to anode, and cations to cathode.
- Where there is more than one possible reaction at each electrode, determine which will occur. Write the half-equation for the reaction at each electrode, showing electrons released at the anode in oxidation and taken up at the cathode in reduction.
- Balance the electrons lost and gained at the anode and the cathode, then add the two half-equations to write the equation for the net reaction.
- Consider what changes would be observed in the cell as a result of the redox processes occurring. These may include colour changes in the electrolyte, precipitation of solid, gas discharge, or No changes.
Electrolysis of water
- The addition of ions increases its conductivity, so usually some ionic compound such as NaOH is added when this electrolysis is performed.
- 1) Ions present: NaOH(aq) → Na+(aq) + OH–(aq)
- 2) At the cathode, possible reactions are:
Na+(aq) + e– → Na(s) E * = –2.71 V
2H2O(l) + 2e– → H2(g) + 2OH–(aq) E * = –0.83 V
- So H2O is preferentially reduced and H2(g) will be discharged.
- At the anode, possible reactions are:
4OH–(aq) → 2H2O(l) + O2(g) + 4e– –E * = –0.40 V
2H2O(l) → 4H+(aq) + O2(g) + 4e– –E * = –1.23 V
- 3) The overall balanced equation is: 2H2O(l) → 2H2(g) + O2(g)
- 4) The observed changes at the electrodes will be:
- colourless gas evolved at both anode (O2) and cathode (H2)
- ratio by volumes of the gases is 2 H2 : 1 O2 (by application of Avogadro’s law on gas volumes)
- the pH at the anode will decrease as H+ is released, while the pH at the cathode will increase as OH– is released.
Factors affecting the amount of product in electrolysis
- Michael Faraday, said that the amounts of products at the electrodes depend on the quantity of electric charge passed through the cell.
- We can quantify electric charge as follows:
- charge = current × time
So: Charge, Q measured in coulomb (C), depends on the current, I measured in amperes (A) and the time, t measured in seconds (s). - The charge carried by one mole of electrons, known as a Faraday (F), is 96 500 C.
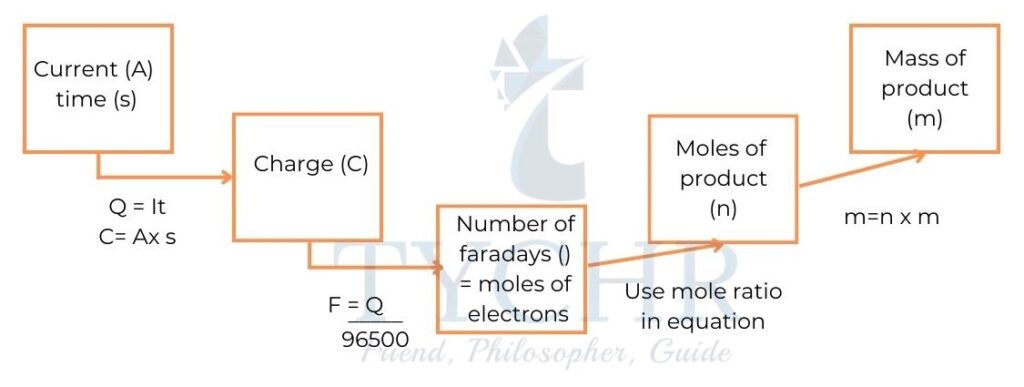
Electroplating: a widely used application of electrolysis
- During the electrolysis of CuSO4(aq), we saw that Cu(s) is deposited on the cathode when either graphite or copper electrodes are used.
- This is one example of electroplating, the process of using electrolysis to deposit a layer of a metal on top of another metal or other conductive object.
- An electrolytic cell used for electroplating has the following features:
- an electrolyte containing the metal ions which are to be deposited;
- the cathode made of the object to be plated;
- sometimes the anode is made of the same metal which is to be coated because it may be oxidized to replenish the supply of ions in the electrolyte.
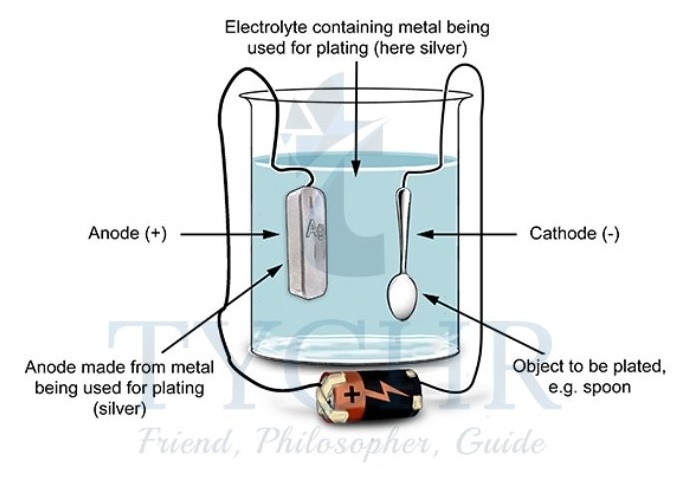
- Electroplating serves many different purposes, some of which are outlined here.
- Decorative purposes. For example, covering a metal with a layer of a more expensive or decorative metal, such as silver- and nickel-plating of cutlery.
- Corrosion control. For example, iron with a layer of zinc deposited on its surface, known as galvanized iron, is protected from corrosion as the zinc will be preferentially oxidized. This is sometimes called sacrificial protection.
- Improvement of function. For example electroplating with chromium improves the wear on steel parts such as crankshafts and hand tools.